Manuel Zimmer is an Adjunct Investigator at the IMP and Professor at the University of Vienna. You find more detailed information about his work and research group at: https://neurodevbio.univie.ac.at/zimmer-research/
Neural Network Dynamics and Behaviour
In the Zimmer lab, we are interested in how neural network dynamics in the brain represent sensory information and perform computations to generate decisions and subsequent behaviours. Moreover, we aim to explain fundamental properties of neuronal circuits, for example the need to sleep. These are key problems in neuroscience, each of which have alone challenged worldwide communities of experts for decades. We, however, propose that a holistic approach should be undertaken to understand these functions in their full context. To make this goal achievable, we take advantage of the uniquely experimentally tractable model organism C. elegans, a 1mm long nematode worm that can be found dwelling in soil. C. elegans has a small nervous system of only 302 neurons with a completely mapped connectome. Despite the small size, it can produce sophisticated behaviours. In recent years, we developed new approaches to quantify C. elegans behaviour in unprecedented detail and to record the activity of all neurons simultaneously in real time. These new technologies, together with the rich and efficient genetic toolkit available for the worm, will allow the first complete understanding of any nervous system’s operational principles. In the long term our holistic approach will enable us to generate a realistic in silico simulation of the brain’s properties and behaviours. We will provide a basic proof of principle working model to guide the study of higher nervous systems and the design of brain inspired computational devices.
Neuronal population dynamics in C. elegans
We developed a nuclear localised calcium indicator protein that together with fast volumetric fluorescence microscopy enables us to measure brain wide activity in real time and at single cell resolution (Movie, Figure 1A) (Schrödel et al., 2013, Nature Methods).
Movie. Pan-neuronal labelling of a C. elegans brain with a fluorescent Ca2+-indicator. Shown is a movie of maximum intensity projections from volumetric whole brain fluorescence Ca2+-imaging. The example recording is from a worm during the lethargus stage, during which animals are tired and spontaneously fall asleep, given that they reside in their comfortable milieu of 10% ambient oxygen concentrations. At 6 min recording time the animal is aroused by atmospheric oxygen concentrations (21%), which rapidly wakes it up. Upon return to 10% oxygen, the animal eventually falls back to sleep.
A milestone discovery was that most of the active neurons in the awake brain coordinate amongst each other to form collective global activity patterns, even in the absence of any sensory stimulus. Brain states with characteristic neuronal activity pattern are repeated in a cyclical fashion. This can be visualised with computational methods revealing features characteristic of dynamical systems (Figure 1A-B) (Kato et al., 2015, Cell). A thorough investigation of these neuronal population dynamics showed that they correspond to action commands and their assembly into a behavioural command sequence (Figure 1B) (Kato et al., 2015, Cell). Our findings impact the current understanding of the principles of neural network function. First, neural networks are not simple input-output devices but exhibit rich intrinsic dynamical properties that interact on par with external inputs. Second, while individual neurons can encode for detailed and important aspects of motor actions, the high-level and long-timescale organisation of behaviour, i.e. the assembly of action sequences, emerges collectively from the network.
Control of sleep and wakefulness
We developed a new paradigm to effectively switch between wakefulness and sleep during whole brain recordings. Animals are alerted and aroused when exposed to atmospheric oxygen levels as they prefer intermediate oxygen concentrations found in their natural habitats. During developmentally timed sleep-prone episodes termed lethargus, they exhibit sleep behaviour at intermediate oxygen levels but can be effectively kept awake by atmospheric oxygen (Figure 1A-B). We found that sleep is a state of brain wide quiescence exempting a set of sleep active neurons. While behaviours in awake animals are represented by dynamic network activity, sleep appears as a stationary fixed point in neuronal state space (Figure 1B). When worms are left unperturbed this sleep state spontaneously emerges, but can be rapidly interrupted by the arousing oxygen stimulus. Our work demonstrates for the first time a global activity signature of sleep in an invertebrate, and it is the first brain-wide single cell resolution sleep study in any animal. Our results support the hypothesis stating that sleep arises via a self-organizing network mechanism; however, the brain exerts control over its global state via sleep active neurons and opposing arousal circuits (Nichols et al., 2017, Science).
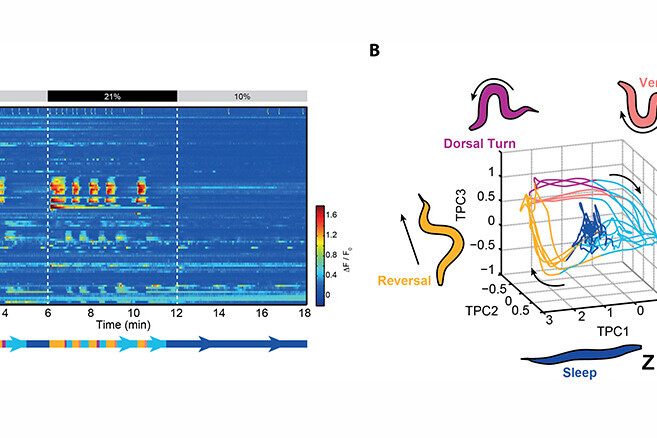
Quantitative behaviour
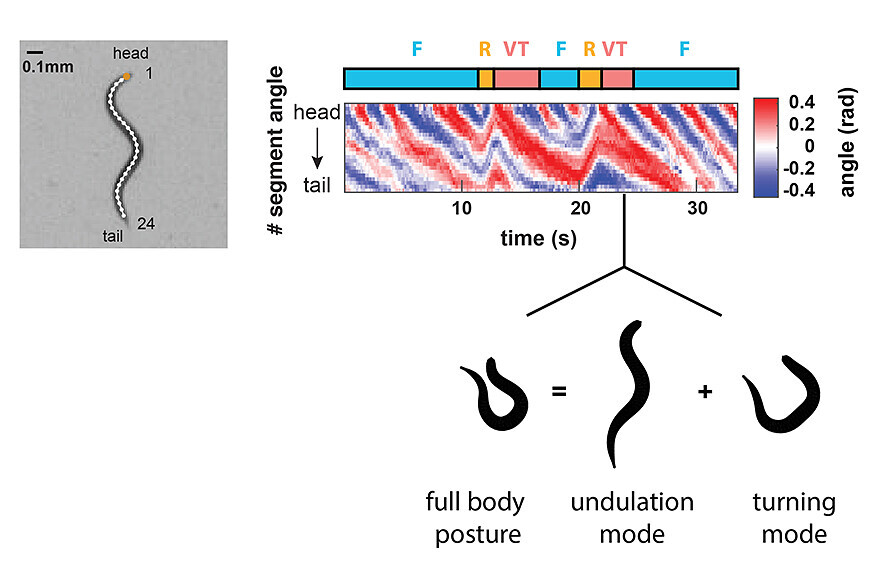
In order to understand brain functions we aim for a comprehensive quantitative description of its behavioural outputs. We developed high throughput behavioural recording techniques to measure the crawling movements of worms in terms of detailed posture time series (Figure 2). Using a computational method based on principal components analysis, we showed that behaviour can be composed out of two elementary motor patterns: the undulation mode contributes to the stereotyped sinusoidal crawling pattern of the animals, while the turning mode contributes to steering and reorientation movements (Figure 2). We identified peptidergic interneurons (termed AVK and DVA) that can gradually control both motor patterns to achieve a spectrum of different behaviours. This work suggests that animals can flexibly generate behavioural diversity out of simple elementary movement modes (Hums et al., 2016, eLife).
Current and Future Research
In our current and future work, we combine advanced microscopy techniques for whole brain and whole nervous system imaging with quantitative behaviour, molecular genetics and computational neuroscience. Our research objectives remain focused on brain dynamics crucial for nervous system function. We aim to understand the information flow from sensory inputs via decision making to behavioural output. We also aim to solve why such a system requires a sleep-wake cycle. Finally, our findings will be recapitulated in a realistic in silico simulation of brain dynamics and behaviour. This holistic approach will enable us for the first time to understand the operational principles of the worm’s nervous system, which we propose are generalisable to larger animals. We are focusing on a few major objectives:
- To develop new microscopy approaches for whole nervous system imaging in freely behaving worms.
- Using whole brain imaging combined with complex sensory stimulation protocols, we are studying how sensory circuits represent features of the environment and interact with brain dynamics to control behaviour. This approach will uncover the neural basis of computations and decision making.
- Using graph theory, we are analysing the worm’s connectome to inform targeted optogenetic manipulations of its nervous system. Here we aim to uncover the mechanisms underlying neuronal population dynamics and to what extent neuroanatomy, i.e. network architecture, can predict neuronal functions.
- While neuronal population dynamics in worms represent a global framework for long timescale action sequences, it remains to be addressed how these action commands are transformed into individual actions and movement patterns at the motor periphery.
- How are whole brain dynamics affected by sleep deprivation and subsequent rebound sleep? Studying sleep homeostasis will shed light on a major mystery: why do animals need to sleep?
- We will reverse engineer the brain in a faithful comprehensive network simulation; the first one available for any complete nervous system. In collaboration with computer scientists, we will leverage this computational platform to design novel brain inspired robotics algorithms and devices.
Selected Publications
- Uzel K, Kato S, Zimmer M. (2022). A set of hub neurons and non-local connectivity features support global brain dynamics in C. elegans. Current Biology. Jul 1:S0960-9822(22)01001-6. doi: doi.org/10.1016/j.cub.2022.06.039. Epub ahead of print. PMID: 35809568.
- Riedl J, Zimmer M. (2022). Tyraminergic corollary discharge filters reafferent perception in a chemosensory neuron. Current Biology. Jun 3:S0960-9822(22)00854-5. doi.org/10.1016/j.cub.2022.05.051. Epub ahead of print.
- Kaplan HS, Salazar Thula O, Khoss N, Zimmer M (2020). Nested neuronal dynamics orchestrate a behavioral hierarchy across timescales. Neuron. 105(3), 562–576.e9. doi.org/10.1016/j.neuron.2019.10.037
- Nichols ALA, Eichler T, Latham R, Zimmer M (2017). A global brain state underlies C. elegans sleep behavior. Science 356, eaam6851. doi.org/10.1126/science.aam6851
- Kato S*, Kaplan HS*, Schrödel T*, Skora S, Lindsay TH, Yemini E, Lockery S, Zimmer M (2015). Global Brain Dynamics Embed the Motor Command Sequence of Caenorhabditis elegans. Cell, 163(3), 656–669. doi.org/10.1016/j.cell.2015.09.034* Authors with equal contribution